Superconductivity: Theory and Applications of a Quantum Phenomenon
This comprehensive article explores the fascinating phenomenon of superconductivity, delving into its theoretical foundations, the mechanisms behind it, and its diverse applications in technology and science. From the first discoveries to modern advancements, superconductivity remains a pivotal area of research with significant implications for the future.

INDC Network : Science : Superconductivity: Theory and Applications of a Quantum Phenomenon
Introduction : Superconductivity is one of the most intriguing phenomena in condensed matter physics, characterized by the complete absence of electrical resistance in certain materials when cooled below a critical temperature. This remarkable property not only defies classical physics but also opens up a world of technological possibilities, ranging from highly efficient electrical systems to revolutionary advances in medical imaging and quantum computing. In this article, we will delve into the theoretical underpinnings of superconductivity, explore its various applications, and discuss the current state of research in this fascinating field.
Understanding Superconductivity
Historical Background : The journey into superconductivity began in 1911 when Dutch physicist Heike Kamerlingh Onnes discovered that mercury exhibited zero electrical resistance when cooled to temperatures near absolute zero (around 4.2 Kelvin). This groundbreaking discovery led to a flurry of research aimed at understanding the underlying mechanisms of superconductivity.
In the decades that followed, various materials were identified as superconductors, including lead, tin, and niobium. However, the theoretical explanation for superconductivity remained elusive until the 1950s when John Bardeen, Leon Cooper, and Robert Schrieffer developed the BCS theory (named after their initials). This theory provided a comprehensive framework for understanding conventional superconductivity and laid the groundwork for future research.
Key Characteristics of Superconductors : Superconductors are defined by two key phenomena:
-
Zero Electrical Resistance: When a material transitions into the superconducting state, it can conduct electricity without any energy loss due to resistance. This allows for the flow of electric current with perfect efficiency.
-
Meissner Effect: Superconductors exhibit the Meissner effect, a phenomenon where they expel magnetic fields from their interior upon entering the superconducting state. This results in the complete levitation of magnets above superconductors, a striking demonstration of their unique electromagnetic properties.
Types of Superconductors : Superconductors can be broadly categorized into two main types based on their behavior in response to magnetic fields and their underlying mechanisms:
1. Type I Superconductors : Type I superconductors are characterized by their complete expulsion of magnetic fields (perfect diamagnetism) and a single critical magnetic field strength. When the applied magnetic field exceeds this critical value, the material transitions back to a normal state. Type I superconductors are typically elemental metals, such as lead and mercury, and are relatively rare.
2. Type II Superconductors : Type II superconductors, in contrast, allow magnetic fields to partially penetrate their interior, creating a mixed state. These materials have two critical magnetic field strengths: a lower critical field (where the material enters the mixed state) and an upper critical field (where the material reverts to the normal state). Type II superconductors include many metallic compounds and alloys, such as niobium-titanium and high-temperature superconductors (HTS).
Theoretical Foundations of Superconductivity
BCS Theory : The Bardeen-Cooper-Schrieffer (BCS) theory provides a microscopic explanation for superconductivity in conventional superconductors. According to this theory, the key mechanism behind superconductivity is the formation of Cooper pairs—pairs of electrons that are bound together at low temperatures.
-
Electron-Phonon Interaction: In a superconductor, electrons interact with lattice vibrations (phonons) in the material. This interaction leads to an attractive force between electrons, allowing them to form Cooper pairs. Unlike ordinary electrons, which repel each other due to their negative charge, Cooper pairs can move through the lattice without scattering, thus enabling zero resistance.
-
Condensate State: The formation of Cooper pairs leads to the emergence of a collective ground state known as the condensate state. In this state, the pairs of electrons condense into a single quantum mechanical wave function that describes their collective behavior. This coherence across the material allows for the supercurrent to flow without resistance.
-
Energy Gap: The BCS theory also predicts the existence of an energy gap in the electronic density of states at the Fermi level. This energy gap represents the energy required to break apart a Cooper pair and return to the normal state. The presence of this gap is a signature characteristic of superconductors.
High-Temperature Superconductivity : The discovery of high-temperature superconductors (HTS) in the 1980s marked a significant milestone in superconductivity research. Unlike conventional superconductors, which require cooling to near absolute zero, HTS can exhibit superconductivity at temperatures above the boiling point of liquid nitrogen (around 77 Kelvin).
The first HTS was discovered in a copper-oxide (cuprate) compound, and subsequent discoveries revealed that a wide range of materials, including iron-based superconductors, also exhibited high-temperature superconductivity. However, the underlying mechanisms behind HTS remain a subject of intense research and debate, as traditional BCS theory does not fully explain their behavior.
-
Electron Correlations: One of the leading theories posits that strong electron-electron correlations play a crucial role in the superconducting behavior of HTS materials. These correlations may lead to the formation of Cooper pairs through mechanisms distinct from the electron-phonon interactions described by BCS theory.
-
Quantum Fluctuations: Some researchers propose that quantum fluctuations in the lattice structure of HTS materials may facilitate the pairing of electrons, resulting in superconductivity at higher temperatures.
-
The Role of Doping: Doping—introducing impurities into the material—can significantly influence the superconducting properties of cuprates and other HTS materials. Understanding how doping affects the pairing mechanisms is an active area of research.
Applications of Superconductivity
Superconductivity has a wide array of applications across various fields, ranging from medical imaging to energy storage. Below, we explore some of the most notable applications of superconductivity:
1. Magnetic Resonance Imaging (MRI) : MRI is a widely used medical imaging technique that relies on superconducting magnets to generate strong and stable magnetic fields. Superconducting materials enable the construction of powerful magnets that produce high-resolution images of soft tissues within the human body. The use of superconductors in MRI machines improves image quality and reduces the overall size of the equipment.
2. Maglev Transportation : Superconductors play a crucial role in magnetic levitation (maglev) transportation systems, where trains can levitate above tracks using magnetic forces. This technology reduces friction, allowing for faster and more efficient travel. Superconducting magnets are employed to create the necessary magnetic fields for levitation and propulsion, leading to significant advancements in high-speed rail systems.
3. Energy Storage Systems : Superconducting magnetic energy storage (SMES) systems utilize superconductors to store electrical energy in the form of magnetic fields. SMES devices can release stored energy quickly and efficiently, making them valuable for stabilizing electrical grids, managing power fluctuations, and supporting renewable energy sources. Their rapid response capabilities make SMES systems an essential component in modern energy management.
4. Particle Accelerators : Superconductivity is instrumental in the construction of particle accelerators, such as the Large Hadron Collider (LHC). Superconducting magnets are used to generate the strong magnetic fields required to steer and focus particle beams at high energies. The use of superconducting materials allows for greater efficiency and higher performance in these cutting-edge research facilities.
5. Quantum Computing : Superconducting qubits are a leading approach in the development of quantum computers. By leveraging the unique properties of superconductors, researchers can create qubits that exhibit quantum behavior, enabling the execution of complex computations beyond the capabilities of classical computers. Superconducting qubits are already being used in several experimental quantum computing systems.
6. Telecommunications : Superconductors are being explored for applications in telecommunications, particularly in the development of high-speed, low-loss transmission lines. The use of superconducting materials can enhance signal quality and reduce energy loss in communication systems, contributing to faster and more efficient data transmission.
Challenges and Future Directions
1. Material Limitations : Despite the promise of superconductivity, challenges remain in finding and developing materials that exhibit superconducting properties at higher temperatures. Most superconductors still require cooling to very low temperatures, making their practical applications expensive and energy-intensive. Research efforts are focused on discovering new materials and improving existing ones to achieve room-temperature superconductivity.
2. Understanding High-Temperature Superconductivity : The mechanisms behind high-temperature superconductivity are not yet fully understood. Ongoing research aims to unravel the complexities of HTS materials, including the role of electron correlations, lattice dynamics, and doping effects. A deeper understanding of these mechanisms could pave the way for the development of new superconducting materials.
3. Scalability and Integration : For many applications, especially in quantum computing and energy storage, scalability and integration of superconducting technologies pose significant challenges. Researchers are exploring innovative approaches to integrate superconductors into existing systems while ensuring reliable performance and cost-effectiveness.
4. Environmental Considerations : The energy-intensive cooling processes required for traditional superconductors raise environmental concerns. As research advances toward room-temperature superconductors, it is crucial to consider the environmental impact of manufacturing and operating superconducting systems. Sustainable practices and materials will be essential in developing a more environmentally friendly superconductivity landscape.
Conclusion : Superconductivity is a captivating quantum phenomenon that has far-reaching implications for both fundamental physics and practical applications. From its discovery over a century ago to the ongoing quest for room-temperature superconductors, the field of superconductivity continues to evolve, pushing the boundaries of our understanding of matter and energy.
As researchers delve deeper into the theoretical foundations and explore novel materials, superconductivity holds the potential to revolutionize technology in diverse areas, including healthcare, transportation, energy management, and computing. The journey into the world of superconductivity is far from over, and as we unravel its mysteries, we move closer to harnessing the full power of this extraordinary phenomenon.
What's Your Reaction?
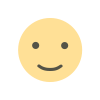
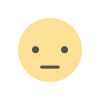
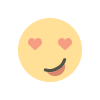
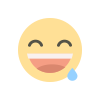
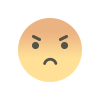
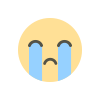
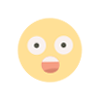